How To Determine The Slowest Step In A Reaction
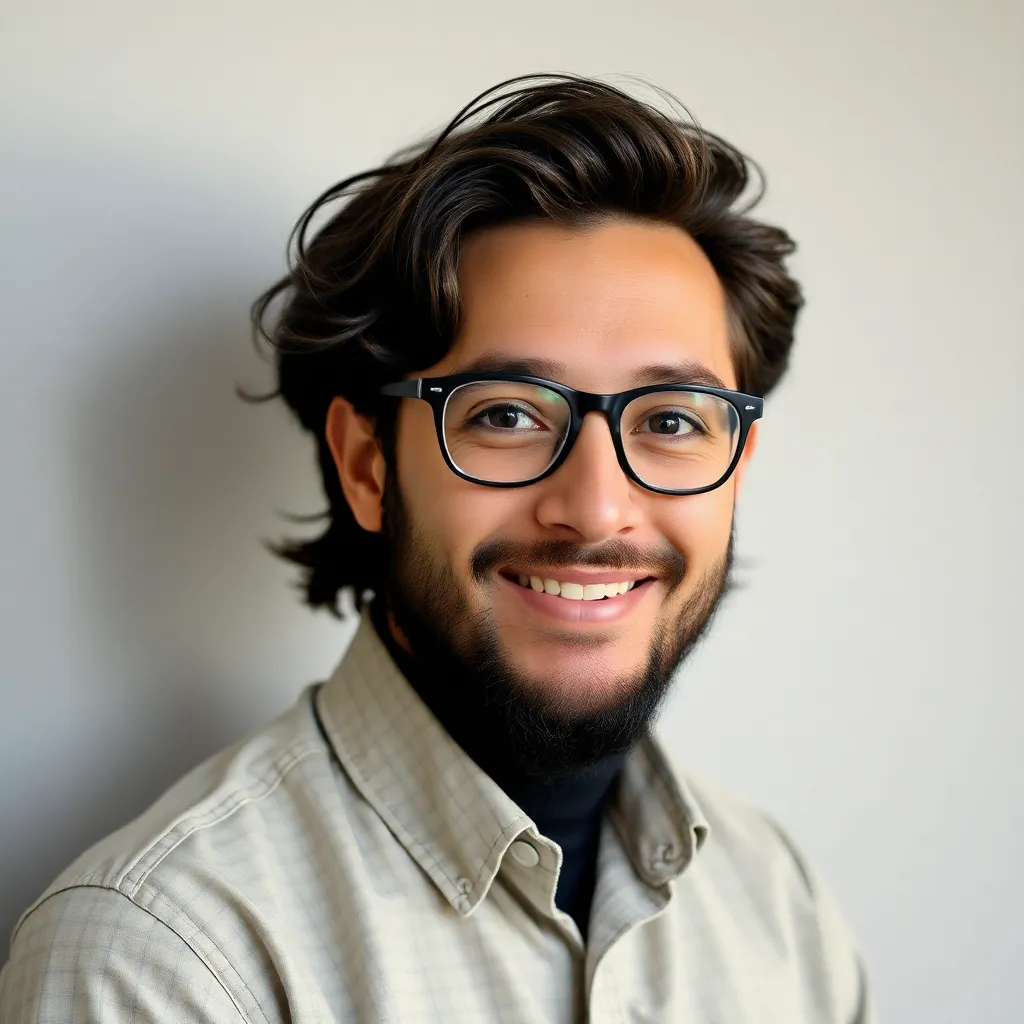
listenit
May 09, 2025 · 6 min read
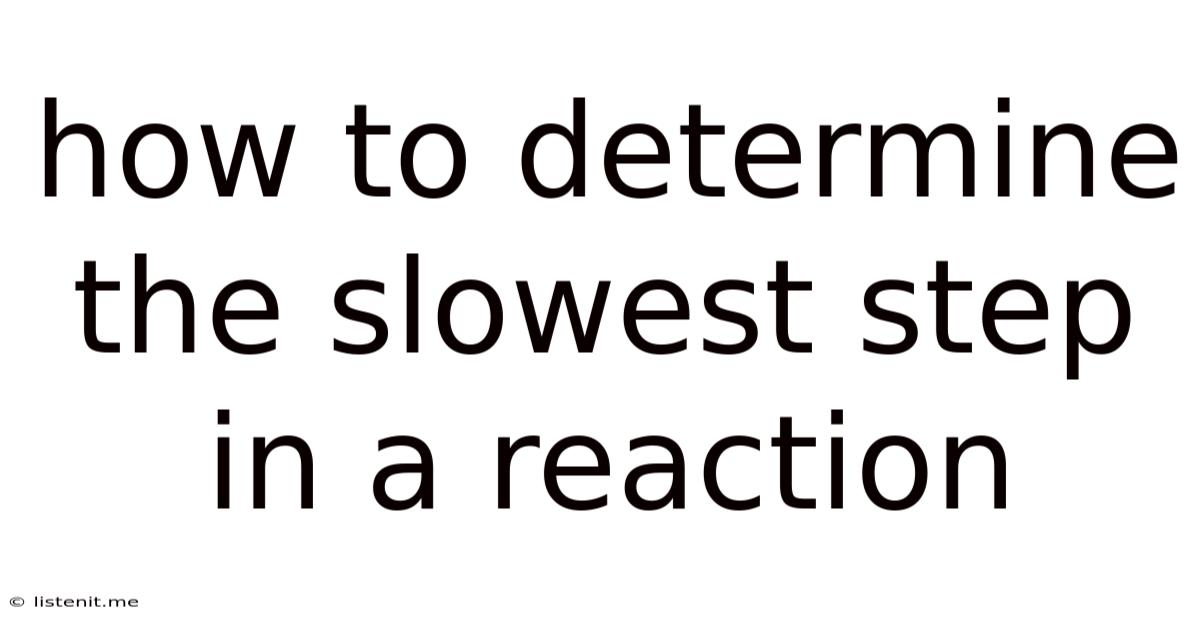
Table of Contents
How to Determine the Slowest Step in a Reaction: A Comprehensive Guide
Determining the slowest step in a chemical reaction, also known as the rate-determining step (RDS) or rate-limiting step, is crucial for understanding reaction kinetics and mechanisms. The RDS dictates the overall rate of the reaction, as the faster steps have little impact on the overall speed if one step is significantly slower. This article will delve into various methods and techniques used to identify the RDS, providing a comprehensive understanding for students and researchers alike.
Understanding Reaction Mechanisms and Rate Laws
Before we dive into identifying the RDS, let's establish a foundational understanding of reaction mechanisms and rate laws. A reaction mechanism describes the sequence of elementary steps that a reaction proceeds through. Each elementary step involves a single molecular event, such as bond breaking or bond formation. The overall reaction is the sum of these elementary steps.
The rate law expresses the relationship between the reaction rate and the concentrations of reactants. It is experimentally determined and generally doesn't directly reflect the stoichiometry of the overall reaction. For example, consider a simple reaction:
A + B → C
The rate law might be:
Rate = k[A][B]
where:
- Rate: is the speed of the reaction.
- k: is the rate constant (temperature-dependent).
- [A] and [B]: are the concentrations of reactants A and B.
This rate law indicates a second-order reaction, meaning the rate is proportional to the concentration of both A and B. The exponents in the rate law (orders of the reaction with respect to each reactant) are experimentally determined, not predicted from the stoichiometry.
Methods for Determining the Rate-Determining Step
Several methods exist for determining the RDS. The choice of method depends on the complexity of the reaction and the available data.
1. Experimental Determination using Initial Rates
This classic method involves measuring the initial rate of the reaction under different initial concentrations of reactants. By systematically varying the concentration of each reactant while keeping others constant, we can determine the order of the reaction with respect to each reactant. This is done by observing how changes in concentration impact the initial reaction rate.
Example:
If doubling the concentration of reactant A doubles the initial rate, the reaction is first-order with respect to A. If doubling the concentration of A quadruples the initial rate, the reaction is second-order with respect to A. By performing this for all reactants, we obtain the rate law. The rate law then gives clues about the RDS. For instance, if the rate law only includes one reactant, that reactant likely participates in the RDS.
Limitations: This method requires precise experimental measurements and careful control of experimental conditions. It's also limited to reactions with relatively simple mechanisms.
2. Analysis of Reaction Intermediates
A reaction intermediate is a species formed during the reaction but consumed before the final product is formed. The concentration of intermediates is generally low and difficult to measure directly. However, spectroscopic techniques (e.g., UV-Vis, NMR) or other indirect methods can sometimes be employed to detect intermediates.
If an intermediate's concentration significantly impacts the reaction rate, it's a strong indicator that the step involving that intermediate is the RDS. A build-up of an intermediate might indicate a bottleneck in the reaction pathway.
Limitations: Detecting and quantifying intermediates can be challenging, particularly for fast reactions.
3. Steady-State Approximation
The steady-state approximation is a powerful technique used to simplify complex reaction mechanisms. It assumes that the concentration of any intermediate remains relatively constant during the reaction. This simplifies the mathematical analysis, allowing for the derivation of a rate law that can provide insights into the RDS.
This approximation is valid when the rate of formation of an intermediate is approximately equal to its rate of consumption. This allows us to write an algebraic equation that relates the concentrations of reactants, intermediates, and products. This equation can then be used to derive the rate law for the overall reaction. The rate law derived often points directly to the RDS.
Limitations: The validity of the steady-state approximation depends on the relative rates of formation and consumption of intermediates. It might not be accurate for reactions with very short-lived or highly reactive intermediates.
4. Kinetic Isotope Effects (KIEs)
KIEs involve replacing an atom in a reactant molecule with its heavier isotope (e.g., deuterium instead of hydrogen) and observing the impact on the reaction rate. A significant change in the reaction rate indicates that the bond involving the substituted atom is broken or formed in the RDS.
This is particularly useful for reactions involving proton transfer or bond breaking steps. If a substantial KIE is observed, it strongly suggests that the step involving the isotopically substituted bond is rate-determining.
Limitations: KIE measurements require specialized techniques and can be expensive.
5. Computational Methods
Modern computational chemistry allows the simulation of reaction mechanisms and calculation of energy barriers for each elementary step. The step with the highest activation energy corresponds to the RDS. Sophisticated software packages are used to perform these calculations, offering insights into reaction pathways and activation energies of each step.
Limitations: Computational methods require sophisticated software, considerable computational resources, and expertise in computational chemistry. The accuracy of the results depends on the quality of the theoretical models used.
Examples Illustrating RDS Determination
Let's examine a few examples to illustrate the application of these methods.
Example 1: SN1 Reaction
The SN1 (Substitution Nucleophilic Unimolecular) reaction involves a two-step mechanism:
- Slow step: Formation of a carbocation intermediate (rate-determining).
- Fast step: Nucleophilic attack on the carbocation.
The rate law for an SN1 reaction is first-order: Rate = k[alkyl halide]. This directly points to the unimolecular ionization step (step 1) being rate-determining.
Example 2: SN2 Reaction
The SN2 (Substitution Nucleophilic Bimolecular) reaction is a concerted mechanism involving a single step:
- Single step: Nucleophilic attack on the alkyl halide, leading to simultaneous bond breaking and bond formation.
The rate law for an SN2 reaction is second-order: Rate = k[alkyl halide][nucleophile]. The reaction's bimolecular nature shows that both reactants are involved in the single, rate-determining step.
Example 3: A More Complex Reaction
Consider a hypothetical three-step reaction:
A + B → I1 (slow) I1 + C → I2 (fast) I2 → P (fast)
where:
- A, B, C are reactants
- I1, I2 are intermediates
- P is the product.
The first step is significantly slower than the other two, hence it's the rate-determining step. The overall rate law would likely reflect the first step's stoichiometry and be dependent on [A] and [B].
Conclusion
Identifying the rate-determining step is fundamental to understanding reaction kinetics and mechanisms. The methods outlined above offer diverse approaches for this crucial task, ranging from simple experimental techniques to sophisticated computational modeling. The choice of method depends on the complexity of the reaction, available resources, and desired level of detail. By carefully analyzing the rate law, intermediate concentrations, isotope effects, or computational simulations, researchers can pinpoint the bottleneck in a reaction pathway, gaining invaluable insight into its behavior and potential for optimization. This understanding is crucial not only for academic purposes but also for designing efficient industrial processes and developing new catalysts.
Latest Posts
Latest Posts
-
To The Power Of One Half
May 09, 2025
-
What Happens To Blue Litmus Paper In Acid
May 09, 2025
-
What Is The Units For Force
May 09, 2025
-
Write 25 As A Fraction In Simplest Form
May 09, 2025
-
Enter The Formula For The Compound Barium Oxide
May 09, 2025
Related Post
Thank you for visiting our website which covers about How To Determine The Slowest Step In A Reaction . We hope the information provided has been useful to you. Feel free to contact us if you have any questions or need further assistance. See you next time and don't miss to bookmark.